Revision – TEV2020 – Perspective
Extracellular vesicle therapeutics: the issue of one size fits all
DOI: https://doi.org/10.47184/tev.2021.01.04Extracellular vesicles (EV) are mediators of intercellular communication locally in tissue microenvironments and enable distal across organ communication between cells of the same origin and those from different sources. EV surface proteins and lipids enable interaction with particular cells, whereas their internal payload of RNA, transcription factors, DNA, enzymes and metabolites functionally alters recipient cells. EV-interactions and uptake induce changes in cellular proliferation, differentiation, cell movement, as well as transcriptional and epigenetic regulation. These unique properties of EV poise them as attractive therapeutics in a broad range of pathologies, but questions remain in translating EV discoveries to effective therapies. Here, I briefly discuss the need for more stringent considerations for EV-therapeutic effects with a focus on EV biodistribution profiles in appropriate disease models and routes of EV administration with a particular focus on the vasculature.
Keywords: Exosome therapy, lipid nanocarrier, biodistribution
Background
Extracellular vesicles (EV) mediate cellular communication locally in tissue microenvironments [1], distally from their cell source following sterile injury such as stroke [2], acute myocardial infarction [3, 4] brain injury [5] and in isolated cells in vitro [6]. The ability of EV to modulate the behaviour and phenotype in recipient cells poises them as an attractive therapeutic. EV therapeutic responses are driven by EV-specific cargo, such as proteins [6], lipids [7], small noncoding RNAs [4], mRNA, transcription factors [8], DNA, metabolites, and enzymes encased within the EV or decorated on the EV surface. For this reason, bioengineering approaches may allow the modification of EV-surface proteins and lipids to enable cellular targeting and strategies to load EV with specific RNA, DNA and pharmacological agents to enable EV as vectors for therapeutics [9]. Thereby, functionally manipulating recipient cells, for example to perturb pro-inflammatory signalling, inhibit cellular proliferation, differentiation and to promote regeneration and repair in a broad range of pathological tissues. There are important considerations when designing EV-therapeutics, such as biodistribution profiles in appropriate disease models and exploration of different methods of delivery, which are possibly, overlooked in current EV research investigations.
There has been tremendous success in the use of lipid nanoparticles in the administration of the mRNA SARs-CoV-2 vaccine internationally for COVID-19 [10]. Ionised lipids bind to negatively charged mRNA, supported by pegylated lipids, phospholipids, and cholesterol molecules to enable trafficking across the plasma membrane, delivering a payload of mRNA to cells for therapeutic effects. Advances, which enabled lipid nanoparticle technologies for drug/mRNA delivery were developed over several decades [11] but there are limitations. Unmodified lipid nanoparticles are not specific and therefore do not allow targeting of individual cells. When administered intravenously lipid nanoparticles are rapidly delivered to tissues, including the liver and spleen where they can show toxicity. The lipid nanoparticles used for the SARs-CoV-2 vaccine delivery are also not universal. Delivery of new mRNA vaccines will require alterations in the carbon tails of the lipids and ester linkages to enable similarly effective delivery and to prohibit toxicity. However, these modifications alter the lipid nanoparticle biodegradability and biodistribution patterns in vivo [12].
Naturally targeting vectors such as EV, can localise to specific cells, penetrate deep within tissues, easily pass through the cell membrane to deliver therapeutic molecules such as RNA and show no toxicity or immunogenicity [13]. EV have a net negative charge (zeta potential) and therefore are stable in physiological buffers and as they transit in the blood. Their inability to divide and replicate mitigates potential tumourgenic effects and they are stable for prolonged periods of time, which may facilitate practical limitations of lipid nanoparticle drug deliver systems, which require precise storage, such as the storage concerns with the COVID-19 mRNA vaccine Pfizer BioNTech (tozinameran). These EV strengths open new and important opportunities for EV-based therapeutics, with potential to generate autologous EV for personalised medical therapy, which would circumvent issues in biocompatibility and capitalise on EV naturally occurring receptors for targeted effects. However, there are concerns on EV heterogeneity, composition, characterization, and the consequences of functional manipulation of EV for targeted effects. Here, I will briefly discuss the need for more stringent assessment of EV-therapeutic biodistribution profiles in appropriate pathological models and highlight the need to explore appropriate routes of EV administration depending on the target organs with a focus on the vasculature (Figure 1 and Table 1).
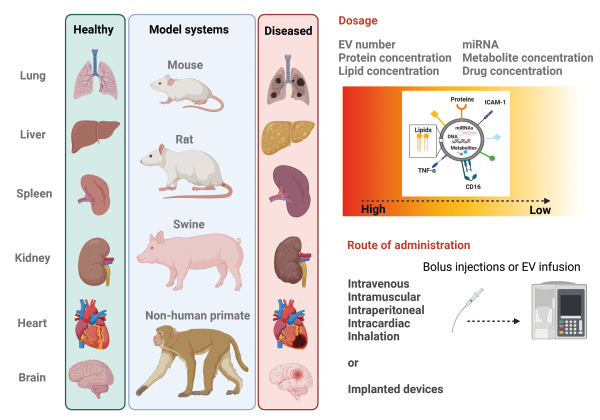
Figure 1: Extracellular vesicle therapeutics: the issue of one size fits all. Extracellular vesicle (EV) biodistribution studies should consider the use of appropriate pathological models in EV therapeutic distribution studies. EV target organs (lung, liver, spleen, kidney, heart and brain) in model organisms (mouse, rat, swine and non human primates) in health (green box) and disease (red box), and explore a range of EV administration methods beyond intravenous injections. These may include intramuscular, intraperitoneal, intracardiac and inhalation routes or delivery of EV through implanted devices. EV dosage should consider EV number, protein lipid, RNA, metabolite and drug concentrations when formulating strategies to perturb pathological signaling in model systems.
Table 1: Summary of the major advantages and disadvantages of Extracellular Vesicle (EV) routes of administration for therapeutic EV.
Route of | Major | Major | Further |
---|---|---|---|
Intravenous | Ease of access and liberation of EV to the peripheral blood | Rapid clearance of EV from the circulation | Major target organs include the lungs, liver, spleen and kidney |
Intramuscular | Localised concentrated delivery of EV | Restricted effects of EV at site of administration | Cardiac injections of EV in preclinical models are often in combination with thoracotomy and infusion using coronary guide wires and stents may yield different effects |
Intraperitoneal | Quick and does not require a high level of technical skill. | Injection of EV into the intestines, bowels, and potential injury to the abdominal organs | Pharmacokinetics of intraperitoneal injections are less well understood |
Intracardiac | Rapid localised injection to the myocardium | Mechanical injury to the heart at site of injection | Quick systemic biodistribution of EV, which enter the ventricles and may have multiple off target effects |
Inhalation | Efficient delivery to the lung mucosal surface | Need for specialised equipment to vaporise EV | Inhalation therapeutics are not well suited for non-respiratory pathologies |
Implanted | Controlled release of EV in afflicted organs | Implantation of medical devices is very invasive | The implanted EV device may require frequent replacement to continually deliver therapeutic effects |
Biodistribution
Intravenous therapeutic EV are attractive because they allow rapid administration with the potential of immediate effects once liberated to the peripheral circulation and delivery to target organs such as the lungs, liver, spleen, kidney, heart, brain, and gastrointestinal tract. But the route of administration, the dose, and the cell source impact the in vivo kinetics of EV [14].
Studies have shown that intravenously injected EV biodistribution profiles are no different to that of intravenously injected liposomes [15], but important considerations are missing. EV are universally and continually produced by cells in the body and their release into the local tissue microenvironments or into the peripheral circulation is controlled by a delicate interplay between biogenesis, release, haemodynamic and mechanical forces [16, 17] acting on the producing and recipient cell. A bolus intravenous injection of EV into the systemic blood pool is likely to behave differently to those released under homeostatic or pathological conditions. It remains largely unknown in most pathological conditions whether repeated administration of therapeutic EV infers better therapeutic benefits or whether it is necessary to continually infuse over a defined time period. Infusion rates for lipid nanoparticles can have differential biodistribution effects and altered toxicity profiles. However, similar infusion studies are missing for EV. It will be important to determine whether infusion rates negatively influence the intended EV therapeutic effects as these can contribute to potential off target effects.
Intravenous injections and infusion rates of EV may be less optimal in individuals with vascular disease, where vascular access for injection is difficult because of non-visible and non-palpable veins. Small vessel disease is a prominent feature in patients with cardiovascular diseases and associated comorbidities such as diabetes and obesity. Vascular disease is associated with elevations in circulating plasma EV and alterations in their composition [18, 19] . In advanced stages of cardiovascular disease, atherosclerotic plaques disturb the natural laminar flow patterns of the blood and that are protective against cardiovascular disease development and thus may alter the in vivo kinetics of injected EV in vessels.
Dosage is an important consideration in EV-therapeutics. Several studies report injections of several hundred micrograms of EV protein into rodent models. Whilst less attention has focused on the use EV concentration, lipid concentration, RNA concentration and in the case of bioengineered EV the payload of encased metabolites and drugs. Extremely high levels of bolus EV injections may enhance blood circulation times and therefore the potential for off target effects, principally in vascular endothelial cells, which avidly uptake EV and show endothelial cell activation and dysfunction [20]. EV-therapeutic studies should report dosage effects, which are comparable to the levels observed in pathological conditions or elucidate why such extraordinary doses are necessary with a focus on potential off target effects by monitoring inflammation and gene expression patterns in non-target organs. If high doses of therapeutic EV are necessary, then infusion systems or implantable devices may be beneficial. Implantable devices mitigate potential harmful effects generated by administration of a more concentrated solution and avoid EV clumping and aggregation, which may be more prone to uptake in the lungs and liver by resident immune cells.
EV target sites, such as the spleen, liver and lungs appear to be ubiquitous amongst EV from almost every cell type and EV from different species [21]. The principal effector cells in EV clearance are macrophages, monocytes and possibly neutrophils. Studies suggest that splenectomy enhances concentrations of circulating peripheral blood EV and that injected EV preferentially accumulate in injured organs and show different biodistribution profiles in healthy animals [22]. In chronic inflammatory pathologies such as dyslipidaemia, obesity, rheumatic diseases, and cardiovascular diseases the liver and spleen can undergo significant alterations in size and exhibit a chronic inflammatory profile. This liver and spleen hyperplasia increases macrophages numbers, which are essential for EV capture from the circulation. However, it remains unknown how these disease specific alterations in EV target sites influence the capture and biodistribution profiles of injected EV. EV clearance from the circulation may be enhanced or perturbed in pathological tissues and therefore the intended biological effects of therapeutic EV may be altered. It is therefore necessary to assess EV therapeutic effects in a range of appropriate biological models. For example, in studies examining therapeutic EV in acute myocardial infarction it may be necessary to show EV biodistribution, EV clearance and EV therapeutic effects in models with comorbidities. Human cardiovascular disease is synonymous with comorbidities such as diabetes, obesity, hypertension, and dyslipidaemia. Therefore, rodent models of diet induced obesity, salt induced hypertension and those that show dyslipidaemia and metabolic dysfunction such as diabetes would add important information on the therapeutic effects of EV. But it is difficult to assess these rapid in vivo kinetic effects without reliable methods for assessment of EV biodistribution.
EV distribution assessment varies considerably and may include positron emission tomography (PET), magnetic resonance imaging (MRI), radiolabelling [23], bioluminescence [24], metabolic labelling [15], fluorescent imaging [4], gold nanoparticles [25], ex vivo gamma counting and miRNA-labelling [4, 26]. Each of these methods have a number of advantages and disadvantages. For example, they allow assessment of EV biodistribution profiles in vivo in real time and under the influence of the systemic circulation, real time evaluation of rapid kinetics, clearance, and organ accumulation (PET, MRI, miRNA labelling) or they allow sub-cellular analysis of EV accumulation deep within tissues (fluorescent imaging). There are major limitations to using dye and fluorescent based methods for EV tracking and biodistribution and these have been comprehensively discussed elsewhere[27]. These EV biodistribution methods inherently influences the overall conclusions drawn from in vivo EV biodistribution studies by providing information on the rapid nature of clearance, organ accumulation and subcellular localisation. Thus a number of EV biodistribution assessment methods should be employed in appropriate disease models to assess tissue clearance and kinetics of therapeutic EV, which may be altered by EV bioengineering approaches or by EV capture by pathological tissues.
The majority of EV biodistribution studies have largely involved small animal models such as the mouse and rat. There is an overall smaller contribution to EV biodistribution in large animal models such as swine [28] and non-human primates. The EV biodistribution profile and intended therapeutic effects in these larger organisms are likely to differ. A single intravenous injection of therapeutic EV consisting of several hundred micrograms of EV protein into a small rodent is a comparatively large payload when compared to studies in swine and non-human primates. Studies examining EV and allometry are required to move into larger animals.
There has been a disproportionate emphasis on intravenous EV-therapeutics and in EV biodistribution assessment. The principal organs in EV capture following intravenous injection are not necessarily the target organs in a broad range of pathologies, such as pancreatic cancer, gastrointestinal disorders, and lymphatic dysfunction. Yet numerous studies report intravenous injections as the go-to source of administration for EV-therapeutics. In our laboratory we focus on the rapid kinetics of immune cells in the splenic reserve following acute myocardial infarction. Our studies have demonstrated a role from endothelial cell derived EV from endothelium as a signal to the remote spleen for the rapid (< 2 hours) induction of neutrophil and monocyte mobilisation [2, 3, 29]. Thus intravenous injections of bioengineered EV allows us to delineate the mechanism and mode of EV-induced cell mobilisation, in a physiological/pathological relevant setting where endothelial cell derived EV from the injured myocardium localise to the spleen. However, intravenous EV-therapeutics aimed at treating organs or tissues other than the lungs, spleen and liver are not well matched and therefore other routes of EV administration must be considered.
Other routes of EV administration
The route of administration affects the biodistribution profiles of EV and investigations should test a number of routes depending on the intended therapeutic effects to ensure maximal delivery and efficiency of therapeutic EV. Other routes of EV administration include intramuscular, intraperitoneal, intra cardiac and retro-orbital injections, intratracheal administration[19], inhalation as aerosols, topical treatment and release of EV through implanted devices (Table 1). These localised routes of administration are often more appropriate for specific pathologies, such as musculoskeletal disorders, regeneration of the heart following myocardial infarction, gastrointestinal inflammation or to reduce overall stress in rodent models. However, it may be necessary to employ several different routes of EV administration to achieve therapeutic effects. Cavallari et al employed intravenous injection of therapeutic EV for immediate effects and complemented this by localised sustained release through intramuscular injections following injury to the hind limb to prevent muscle damage [30].
In cardiovascular diseases, blood vessels can become progressively obstructed by the formation of atherosclerotic plaques, leading to acute myocardial infarction, stroke, and peripheral vascular disease. A primary therapy is the insertion of a stent to restore lumen diameter, blood flow and therefore perfusion of distal tissues. Bare metal stents and drug eluting stents can induce mechanical injury and induce higher rates of stent thrombosis, respectively. Exosome-eluting stents have shown promising results by enhancing endothelium regeneration [31] and reducing the rate of in-stent restenosis. Mesenchymal stem cell derived (MSC) exosome coated stents release a 3-fold higher payload of exosomes in response to reactive oxygen species when compared to physiological conditions [32]. Although the underlying mechanism by which these stent delivered MSC-exosomes mediated, superior patency is unknown. However, these localised anti-inflammatory effects mediated by local delivery of therapeutic EV may not be possible by traditional routes of intravenous injection.
There are many other considerations for EV-therapeutics. EV from different cell sources behave differently in vivo. Therefore, EV surface modifications, which may enable better circulation times or targeting to specific tissue may alter the intended beneficial biological effects of therapeutic EV. For example, surface modification of EV by glycosylation alters in vivo kinetics. O-deglycosylation enzymes enhance delivery to the lungs, whereas N-deglycosylation has no differential effect [33]. Similarly, neuraminidase, which digest sialic residues on glycoproteins, enhances EV delivery to the lungs [34]. However, it remains unknown whether these surface modifications, which enable targeted effects may have better therapeutic effects or more detrimental effects depending on their route of administration.
Conclusion
Liposomes were approved for clinical treatment 34 years after their discovery. EV based therapeutics are promising and there are already registered clinical trials producing GMP certified EV for treatment of a broad set of pathologies. Challenges in translating all potential EV therapeutic findings to clinical trials may be limited by use of healthy rodent models and intravenous injections for delivery of therapeutic EV. A better consensus is needed on EV bio distribution profiles in appropriate disease models for EV based therapeutics, with a particular focus on appropriate modes of EV administration.