Extracellular vesicles in ciliary signalling
DOI: https://doi.org/10.47184/tev.2022.01.07Primary cilia are tiny cellular protrusions deeply involved in intercellular communication. Initially misjudged as a rudiment of motile cilia, it turned out that the primary cilium functions as the cell’s antenna mediating signals which are indispensable for proper human development and homeostasis. Ciliary dysfunction results in severe human diseases collectively referred to as ciliopathies. Originally, ciliopathies were considered to be rare diseases, but the number of diseases identified as or assumed to be ciliopathies is permanently rising. Even common diseases such as cancer or neurodegenerative diseases are considered to be associated with primary cilia. Remarkably, cilia-mediated signalling is associated with extracellular vesicles. In this review, we summarise the current knowledge about the relationship between primary cilia and extracellular vesicles and discuss the role of this relationship in the development of human diseases.
Keywords: Cilia, ciliopathies, intraflagellar transport, BBS4, BBS6
Introduction
In 1675, Antony Van Leeuwenhoek described a living protozoan "with divers incredibly thin feet, or little legs, which were moved very nimbly" [1]. Today, these "thin feet, or little legs" are referred to as cilia. Due to Van Leeuwenhoek’s report, cilia are the oldest known cell organelles. They represent tiny cytoplasmic protrusions with a length of 1-15 μm. A distinction can be drawn between motile and immotile cilia. By their beating, motile cilia act as the driving force of several dynamic processes. Apart from enabling protozoa to move, they generate the nodal flow, create the movement of sperms and transport mucus through the trachea or oocytes through the Fallopian tube [2]. For a long time, immotile cilia were considered to be non-functional vestiges originally derived from motile cilia [3]. In the 1970s, a sensory function of immotile cilia which are also known as primary cilia was suggested. Around the turn of the millennium, several studies confirmed this suggestion [4]. In addition to the sensation of external stimuli such as fluid flow, light or odors, primary cilia mediate numerous signalling pathways which are essential for vertebrate development and homeostasis, such as hedgehog (HH) signalling, Wingless-Int (WNT) signalling, platelet-derived growth factor receptor-α (PDGFRα) signalling and transforming growth factor-β (TGF-β) signalling [5]. The dysfunction of cilia results in severe, often deadly human diseases collectively termed ciliopathies. According to recent estimates, ciliopathies affect between 1:700 and 1:2000 people in the general population worldwide [6]. Importantly, faulty primary cilia cause more varied sensory, physiological and developmental abnormalities than defective motile cilia [7]. In the past, ciliopathies were considered to be rare diseases comprising disorders such as polycystic kidney disease, Meckel–Gruber syndrome, Joubert syndrome, Bardet–Biedl syndrome, Leber congenital amaurosis, Senior–Løken syndrome, orofaciodigital syndrome type 1, Alström syndrome, Jeune asphyxiating thoracic dystrophy, Ellis–van Creveld syndrome and Sensenbrenner syndrome. But since the number of diseases identified as or assumed to be ciliopathies is permanently rising, this point of view has changed. Even common diseases such as cancer or neurodegenerative diseases are associated to primary cilia [5, 8].
In the main, primary cilia are composed of three different compartments – the axoneme, the basal body (BB) and the transition zone (TZ) (Figure 1).
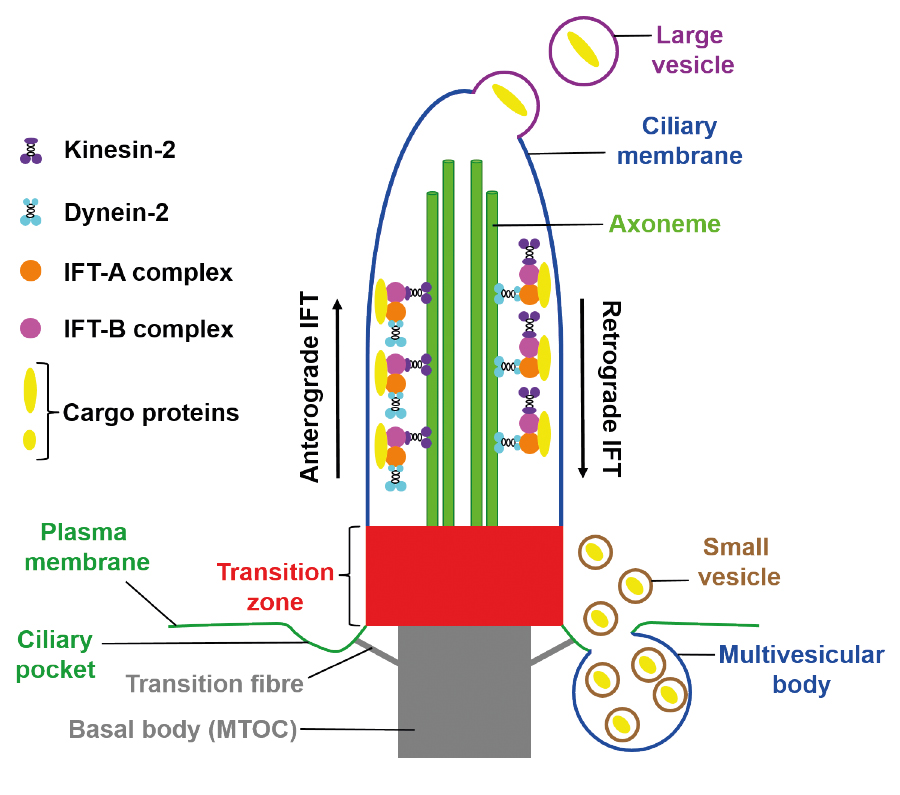
Figure 1: Schematic and simplified illustration of primary cilia structure and of ciliary extracellular vesicle (EV) release. The basal body is anchored in the plasma membrane with the help of transition fibres. It is a modified microtubule organising centre (MTOC) controlling vesicular trafficking. The transition zone acts as the ciliary gatekeeper at the proximal end of the axoneme which grows out of the basal body. For this reason, the composition of the ciliary membrane is different from the composition of the plasma membrane. Proteins that pass the transition zone are transported through the cilium by the intraflagellar transport (IFT). The anterograde IFT is driven by the motor protein kinesin-2 and by the IFT-B complex and carries the cargo proteins from the ciliary base to the ciliary tip. The retrograde IFT is implemented by the motor protein dynein-2 and by the IFT-A complex and brings the cargo proteins from the ciliary tip to the ciliary base. Large ciliary EVs bud off from the ciliary apex, while small ciliary EVs are thought to be launched by multivesicular bodies at the ciliary pocket. EVs have also been found attached along the cilium. In order to keep the clarity of the figure, these EVs are not depicted in this figure.
The axoneme is the microtubule-based scaffold of the cilium and originates from the BB – the modified mother centriole. The nine doublet microtubules of the axoneme are arranged in a ring-like fashion and give stability to the cilium. The transport of proteins within the cilium is referred to as intraflagellar transport (IFT) and is implemented along the axoneme. The anterograde IFT represents the motility of proteins from the base to the tip of the cilium, while the retrograde IFT describes the protein transport back to the ciliary base. The IFT requires the action of motor proteins (kinesin-2 for anterograde IFT and dynein-2 for retrograde IFT) and of so-called IFT proteins (Figure 1). The anterograde IFT is driven by proteins of the IFT-B complex, the retrograde IFT by proteins of the IFT-A complex [9]. The proteins to be transported within the cilium are bound to IFT proteins and the IFT-cargo protein complex is attached to motor proteins which move along the axonemal microtubules. At the very proximal end of the axoneme, a region of about 0.5 μm controls ciliary protein import and export. For this reason, the proximal region of the axoneme – the TZ – governs ciliary protein composition and is known as the ciliary gatekeeper [7]. The TZ consists of several protein complexes and a large number of ciliopathies is caused by mutations in genes encoding TZ proteins [7, 10].
Extracellular vesicles (EVs) represent lipid bi-layered structures released from cells. Their composition depends in a large part on the cell of origin. EVs contain different cargos, such as proteins, lipids and nucleic acids, and are categorised based on their size and source. Small EVs (with a diameter comprised from 30 nm to 100 nm) are referred to as exosomes originated from multivesicular bodies (MVBs). Large EVs (with a size between 50 nm and 1 μm) named ectosomes form by the budding of the plasma membrane [11]. Both small and large EVs are biologically active and transport components known to be involved in intercellular signalling. Previously, investigations in mouse embryos, in the fruit fly Drosophila melanogaster and in mammalian cells revealed that EVs contain active components of HH and WNT signalling [12-14]. In this way, EVs regulate various cellular processes such as cell proliferation, apoptosis, cell growth, cell migration, cell differentiation etc. [15].
Illuminating the relationship between primary cilia and extracellular vesicles
Primary cilia function as the cell’s antennae receiving and mediating signals from their environment to regulate cellular processes, including proliferation, differentiation, migration etc. [16, 17]. After the uptake of proliferative signals, cells leave the quiescent state (G0 phase) and re-enter the cell cycle. Before the mitotic entry, primary cilia are resorbed. Remarkably, ciliary resorption and cell-cycle progression are associated with EVs. In a process referred to as ciliary decapitation cilia tips are excised and released as EVs. Remarkably, tubulin was not detected in the decapitated parts of the cilium indicating that ciliary decapitation could also be defined as an unsheathing of the axoneme. Subsequent to ciliary decapitation, cilia are disassembled and enter the cell cycle [18]. Consequently, the release of EVs can serve as the start signal for the retraction of primary cilia.
However, not every EV release results in ciliary retraction. Several studies highlight the release of ciliary EVs as a mechanism to rapidly discard ciliary proteins [19-24]. These proteins destined to be removed from the primary cilium are encapsulated into EVs that in most cases (especially in mammalian cells) are shed at the ciliary tip. To name this selective process, the term „ectocytosis“ has been coined in 2017 [21]. Importantly, ectocytosis increases when the retrograde IFT is impaired and the intraciliary removal of proteins stagnates [20-24]. This suggests ectocytosis as a safety mechanism to prevent protein overload within primary cilia and also excessive ciliary elongation.
An interesting special case was observed in photoreceptor cells. The cilia of these cells are able to release a huge amount of EVs. However, these EVs do not dispose superfluous proteins. Their release is blocked by the protein peripherin and their membranes are used to build the numerous tightly packed (disc) membranes of the photoreceptor outer segment [25]. As the cilia of photoreceptors are unique organelles which differ from cilia of other cells, the enormous amount of released EVs might be an exception which cannot be found at other cilia [26].
The role of extracellular vesicles in cilia-mediated signalling
Apart from their contribution in driving the cilia cycle and the cell cycle as well as in the disposal of waste and in the formation of photoreceptor membranes, EVs are also involved in cilia-mediated signalling. Accordingly, several reports outlined the attachment of vesicles to the ciliary membrane [27-29]. In this context, it is crucial to distinguish between two different functions of primary cilia in intercellular signalling. Initially, primary cilia were regarded as signal receivers. However, more and more studies provide insights into the role of primary cilia as signal transmitters. These novel insights are based to a large extend on the observations of EVs shed by primary cilia (hereafter named ciliary EVs). The first evidence of vesicles launched by primary cilia came from studies in the green alga Chlamydomonas reinhardtii and in the nematode Caenorhabditis elegans [20, 30, 31]. Moreover, the parasite Trypanosoma brucei rhodesiense, the infectious agent of the East African sleeping sickness, secretes ciliary vesicles which are received by conspecifics in order to transfer resistance to the immune defence of the host [32]. Recently, it was demonstrated that C. elegans communicates from one individual to another by releasing ciliary EVs in a mechanoresponsive manner [33]. Importantly, ciliary EV budding does also take place in mammalian cells [21, 34]. Comparing ciliary EVs with cytosolic EVs, Mohieldin and colleagues discovered different compositions between these two types of EVs [35]. They can be distinguished with regard to protein classes and signalling pathways. This finding demonstrates that the composition does not only depend on the cell of origin but also on the subcellular structure of origin. In line with this conclusion, previous studies detected cilia membrane-specific proteins within EVs [27, 36]. Although the composition of cytosolic EVs and ciliary EVs exhibits differences [35], both EV types are able to mediate signalling pathways. As mentioned above, cytosolic EVs play a role in HH and WNT signalling [12-14], while a very recent study shows the transmission of signalling pathways, such as WNT signalling and TGF-β signalling, via ciliary EVs in mammalian cells. This finding demonstrates the importance of ciliary EV-mediated signalling in mammalian intercellular communication [34]. Furthermore, cilia-mediated signalling can be ceased by releasing active, ciliary membrane-bound receptors of signalling pathways in ciliary EVs [21].
Importantly, ciliary EVs comprise both small and large EVs. While the association between large EVs and primary cilia is obvious, as these EVs are shed by the ciliary membrane (Figure 1), the link between small EVs and primary cilia is still under debate. Volz and colleagues revealed that the dysfunction of primary cilia leads to an increased release of small EVs and to an alteration in their composition [34]. Furthermore, components of different signalling pathways are differentially loaded upon ciliary dysfunction. These findings demonstrate a close relationship between small EVs and primary cilia in which primary cilia control the effect of small EVs on intercellular signalling. It is unknown how primary cilia realise this regulation. Until now, MVBs have not been detected within primary cilia [11]. MVBs seem to be too large to enter the cilium. Consequently, it was suggested that the plasma membrane which surrounds the ciliary base – the ciliary pocket – might be the origin of small EVs [34] (Figure 1).
Regulation of ciliary vesicle release
Not only the role of primary cilia in the release of small EVs but also the regulation of large EV secretion directly from the ciliary membrane is poorly understood. As ciliary EVs are thought to be important for intercellular communication, the mechanisms underlying the release of EVs from primary cilia is currently one of the great topics in cilia research. Several reports tried to shed light on this process but the picture is largely incomplete. Here, we summarise the current knowledge about ciliary EV release.
Both the actin cytoskeleton and the microtubule cytoskeleton play a major role in the secretion of ciliary EVs. During ectocytosis, a coordinated actin machinery involving actin, myosin 6 and drebrin triggers EV launching. In this process, the polymerisation of actin is required [21]. F-actin is also needed for the ciliary decapitation process. Phua et al. revealed that the phosphoinositide 5-phosphatase INPP5E restricts actin polymerisation in primary cilia. When INPP5E leaves the cilium, F-actin is allowed to enter the cilium and accumulates at the site of ciliary decapitation [18].
Extensive investigations in C. elegans uncovered the importance of posttranslational modifications of tubulin in ciliary EV budding. Truncation of the tubulin polyglutamylase tubulin tyrosine ligase like-11B (TTLL-11B) isoform results in a decreased amount of released ciliary EVs in C. elegans [37]. In addition, the truncated tubulin deglutamylase cytosolic carboxypeptidase-1 (CCPP-1) leads to the same effect demonstrating that a tight regulation of ciliary tubulin glutamylation is essential for proper ciliary EV release. Remarkably, the velocity of the IFT motor protein KLP-6 is affected by tubulin glutamylation defects and KLP-6 governs ciliary EV secretion and cargo sorting [31, 37]. In the context of the ciliary microtubule cytoskeleton, the α-tubulin isotype TBA-6 which is crucial for axonemal formation in cephalic male neurons of C. elegans plays an essential role in EV cargo sorting, although its truncation does not alter the velocity of the kinesin motor KLP-6 [38].
A potential link between myristoylation and ciliary EV launching might be reflected by the presence of a putative myristoylation sequence at the N terminus of TTLL-11B [39]. As myristoylation is required for the control of EV secretion by the CILiary localisation protein CIL-7, it is suggested that myristoylation functions as a ciliary EV targeting sequence [11, 37, 40]. Another group of proteins involved in the formation of ciliary EVs belongs to the endosomal sorting complex required for transport (ESCRT). In Chlamydomonas, members of this complex are present at the TZ, in ciliary membranes and in ciliary EVs. They regulate ciliary EV budding and, in this way, ciliary length [41, 42].
The role of ciliary extracellular vesicles in the development of ciliopathies
Signal transduction cascades mediated by primary cilia control cellular processes during the entire human life. Consequently, their proper functioning is essential for human development and health maintenance. The dysfunction of these signalling pathways leads to the occurrence of ciliopathies. Unfortunately, the current treatment of ciliopathies is limited to symptomatic therapies, while a curative medication against ciliopathies is lacking. To develop curative therapies against ciliopathies, it is required to uncover the molecular mechanisms underlying impaired cilia-mediated signalling. In this context, the investigation of ciliary EV biology might be essential. In line with this, a few reports suggested ciliary EVs as potential pathological contributors to e. g. neurological diseases, cancer and cardiovascular diseases [43-45].
Remarkably, a relation between ciliary EVs and a couple of known ciliopathy proteins has already been shown. Components of the IFT are tightly involved in the formation of ciliary EVs. In C. elegans, the deficiency of the IFT-A complex component IFT122/DAF-10 and of the IFT-B complex member IFT-88/OSM-5 cause a decrease in ciliary EV launching [31]. Accordingly, murine cells lacking IFT-88 produce significantly less ciliary EVs [46]. Furthermore, the loss or truncation of BBSome components such as BBS4 and BBS6 in cells from mouse kidneys or BBS10 in patient cells result in increased ciliary EV budding [34]. The BBSome acts as a key player in IFT assembly and stabilises both, the IFT-A and the IFT-B complex. It was formerly suggested that the BBSome governs ciliary EV budding by targeting the G-protein RAB28 to the ciliary membrane [47].
The differences between the amount of ciliary EVs released in the Ift mutant cells and in the Bbs mutant cells could be based on the type of released EVs. While the report studying EV launching in Bbs mutant cells refers to small ciliary EVs [34], the study on EVs released from Ift mutant cells does not differentiate between small and large ciliary EVs [46]. It is conceivable that the analysis of EV budding from Ift mutant cells relates to large ciliary EVs and that this might be the reason for the discrepancy between the two studies. Remarkably, the mentioned differences between the number of launched ciliary EVs seem to correlate with the amount of ciliated cells. While the number of primary cilia is severely reduced in Ift88 and Ift122 cells [31, 46], primary cilia amount increases by BBS4 and BBS6 deficiency [34]. Thus, loss of primary cilia causes loss of ciliary EVs. As the lack of primary cilia leads to the development of ciliopathies, the reduction of ciliary EVs might be one of the reasons for the occurrence of ciliopathies. Although the disruption of the BBSome provokes the formation of more cilia and more ciliary EVs, the deficiency of BBS proteins causes the Bardet-Biedl syndrome. Previously, it was shown that enhanced ectocytosis as observed in Bbs4 and Bbs6 mutant cells results in a decrease of ciliary length [21]. Nevertheless, primary cilia in Bbs4 and Bbs6 mutant cells are elongated [34]. This finding indicates that the increase in ciliary length is caused by other functions of the BBS proteins independently of ciliary EV launching. As ciliary length alterations are indicative for ciliary defects [48], it is conceivable that ciliopathies caused by mutations in BBS4 and BBS6 develop due to ciliary EV-independent mechanisms. However, the protein composition of ciliary EVs is significantly changed in Bbs4- and Bbs6-negative cells [34]. This phenomenon namely an altered ciliary EV protein composition was also described in ciliopathy patients [49] emphasising the necessity for further research to shed light on the relationship between ciliary EVs and the development of ciliopathies.
Conclusion
Primary cilia can be dynamically assembled and disassembled and the protein composition (e. g. the concentration of receptors and signal transduction cascade mediators) within primary cilia is tightly regulated [16]. EVs enhance the mobility of signals in the extracellular space and govern the extracellular amount of signals by endosomal routing, sorting and secretion [50]. The combination of primary cilia and EVs as regulatory units in signalling is an excellent example of fine-tuning concentrations of receptors and ligands at the space of action.
It was already shown that ciliary EVs contribute to the transduction of cilia-mediated signalling thereby participating in intercellular communication [32-34]. But it is yet unclear how important these processes are in intercellular communcation and in the development of diseases. As outlined above, much evidence indicates an eminent importance of ciliary EVs in regulating signalling pathways ensuring proper development and health maintenance. We are just at the beginning of elucidating the meaning of ciliary EVs and of putting the puzzle of findings in different model organisms together. Further studies on ciliary EV biology are a worthwhile purpose as ciliary EVs might be a key for the understanding of molecular causes underlying ciliopathies and bear the potential to serve as biomarkers for disease diagnosis or even as targets to design a curative therapy against ciliopathies.